Authors: William J. Sagues1, Sunkyu Park2, Hasan Jameel1, Daniel L. Sanchez2*
Full citation: Sagues, W. J., Park, S., Jameel, H., & Sanchez, D. L. (2019). Enhanced carbon dioxide removal from coupled direct air capture–bioenergy systems. Sustainable Energy & Fuels, 3(11), 3135-3146.
Abstract: Negative emission technologies (NETs) play a prominent role in climate change mitigation strategies consistent with limiting global warming to well below 2°C. Direct air capture (DAC) and bioenergy with carbon capture and sequestration (BECCS) are the two leading engineered methods of CO2 removal currently under development. Major limitations to scaling DAC and BECCS technologies include high demands for energy and natural resources, respectively. To date, there has been almost no research on the synergies between DAC and BECCS technologies.
BECCS refers to the utilization of renewable biomass resources for energy and the subsequent capture and sequestration of CO2 via injection into geologic formations.1–3 The advantages of using BECCS for engineered CO2 removal include relatively low cost and the existence of established technologies for both biomass conversion and CO2 capture and sequestration.3 The major drawbacks of BECCS technologies are their substantial land requirements, sustainability impacts at scale, limited resource potential, and social barriers to acceptance.4–6 Nonetheless, the US National Academy of Sciences recently classified BECCS as one of four CO2 removal technologies ready for immediate deployment.3 DAC refers to the direct removal of CO2 from the atmosphere and subsequent sequestration. Two leading DAC technology platforms employ liquid solvents and solid sorbents.7,8 Several companies are currently commercializing technologies that use these platforms, including Carbon Engineering, which uses a liquid alkaline solvent for thermal energy-driven calcium looping,9 and Global Thermostat and Climeworks, which use solid-supported amine sorbents for thermal energy-driven adsorption/desorption.10,11 The advantages of using DAC for engineered CO2 removal include flexibility in site location, low land footprint, and potentially limitless scale. However, DAC technologies suffer from high costs, substantial energy requirements, and commercial immaturity.7 The US National Academy of Sciences recently reported the levelized costs of CO2 removal via DAC to be uneconomical in current policy environments.3,12
Many emerging DAC innovators claim that fossilized hydrocarbons, renewable electricity, or nuclear energy will provide the thermal energy necessary for operation.3 Biomass, however, is frequently neglected in such analyses: for instance, recent energy system modelling of DAC integration into low-carbon heat and power systems did not consider biomass as an energy source.13 Biomass combustion technologies hold several advantages over other low-carbon technologies for providing thermal energy for DAC, including high heating efficiencies, high technology readiness, and the ability to contribute to CO2 removal (Figure 1).14,15
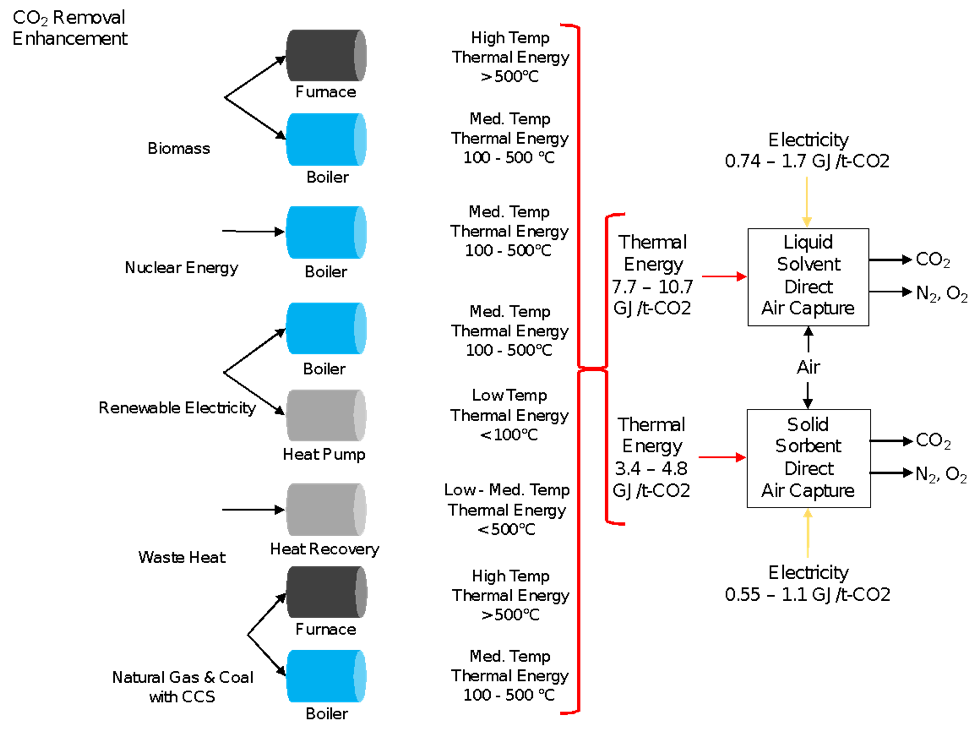
The use of biomass as an energy source for DAC has been largely overlooked in prior literature. Here, we conduct a techno-economic and geospatial analysis to understand the economic and carbon removal potential of coupled BECCS and DAC technologies. The aforementioned limitations to BECCS and DAC technologies are curtailed when coupled into one system. We estimate biomass integrated DAC systems can increase net CO2 removal by 109 – 119% at lower costs than standalone reference systems. If hybrid BECCS-DAC systems were deployed across the US using biomass resources co-located with geologic storage, 1.5 Gt of CO2 could be sequestered annually without long-distance biomass or CO2 transport in 2030. Innovation in the design and implementation of coupled BECCS-DAC systems would have a profound impact on CO2 removal, if realized. However, social acceptance, environmental protection, and appropriate political incentives are necessary for large-scale deployment of CO2 removal systems fueled by biomass.6,9 Fortunately, the US Department of Energy has conducted thorough assessments of sustainable biomass availability to help minimize environmental and social harm as CO2 removal systems continue to advance.24 Historically, the lack of US policies placing a value on CO2 has been a barrier to the deployment of BECCS and DAC technologies, however, the US Congress recently enhanced the Section 45Q carbon oxide sequestration tax credit to help overcome this barrier.34
This analysis takes a significant step forward in the development of NETs by demonstrating the technical and economic viability of coupled BECCS-DAC systems capable of removing significant quantities of CO2 with lower cost and environmental impact. Moving forward, BECCS-DAC systems can drive efficient, cost-effective, and locationally-optimized usage of biomass resources for engineered CO2 removal. As the level of attention towards CO2 removal technology development continues to grow, BECCS-DAC systems deserve serious consideration.
Read Sagues et al.’s complete paper in Sustainabile Energy & Fuels.
References:
1: M. Allen, M. Babiker, Y. Chen, H. de Coninck, S. Connors, R. van Diemen and O. P. Dube, Intergov. Panel Clim. Chang.
2: G. P. Peters and O. Geden, Nat. Clim. Chang., 2017, 7, 619–621.
3: National Academy of Sciences, Natl. Acad. Press, 2018, 131–171.
4: E. Baik, D. L. Sanchez, P. A. Turner, K. J. Mach, C. B. Field and S. M. Benson, Proc. Natl. Acad. Sci., , DOI:10.1073/pnas.1720338115.
5: H. J. Buck, Clim. Change, 2016, 139, 155–167.
6: K. S. Lackner, Science (80-. ).
7: D. Sandalow, J. Friedmann and C. McCormick, Direct Air Capture of Carbon Dioxide: ICEF Roadmap 2018, https://www.icef-forum.org/pdf2018/roadmap/ICEF2018_Roadmap_Draft_for_Comment_20181012.pdf.
8: E. S. Sanz-Pérez, C. R. Murdock, S. A. Didas and C. W. Jones, Chem. Rev., 2016, 116, 11840–11876.
9: D. W. Keith, G. Holmes, D. St. Angelo and K. Heidel, Joule, 2018, 2, 1–22.
10: 2014, No. 14715962.8 (European Patent).
11: 2015, No. 2015/0283501 (US Patent).
12 :C. Bataille, C. Guivarch, S. Hallegatte, J. Rogelj and H. Waisman, Nat. Clim. Chang., 2018, 8, 648–650.
13: F. Creutzig, C. Breyer, J. Hilaire, J. Minx, G. P. Peters and R. Socolow, Energy Environ. Sci., , DOI:10.1039/c8ee03682a.
14: McKinsey & Company, Decarbonization of industrial sectors: the next frontier, https://www.mckinsey.com/~/media/McKinsey/Business Functions/Sustainability and Resource Productivity/Our Insights/How industry can move toward a low carbon future/Decarbonization-of-industrial-sectors-The-next-frontier.ashx.
15: EU Biomass Availability and Sustainability Information System, Report on conversion efficiency of biomass, http://www.basisbioenergy.eu/fileadmin/BASIS/D3.5_Report_on_conversion_efficiency_of_biomass.pdfAhttp://www.basisbioenergy.eu/%0Ahttp://www.basisbioenergy.eu/%0Ahttp://www.basisbioenergy.eu/.
Author affiliations:
1Department of Forest Biomaterials, North Carolina State University, 2820 Faucette Dr., Raleigh, NC 27606, USA.
2Department of Environmental Science, Policy, and Management, University of California-Berkeley, 130 Mulford Hall #3114, Berkeley, CA 94720.
Correspondence and requests for materials should be addressed to Daniel Sanchez: sanchezd@berkeley.edu